METAP Project
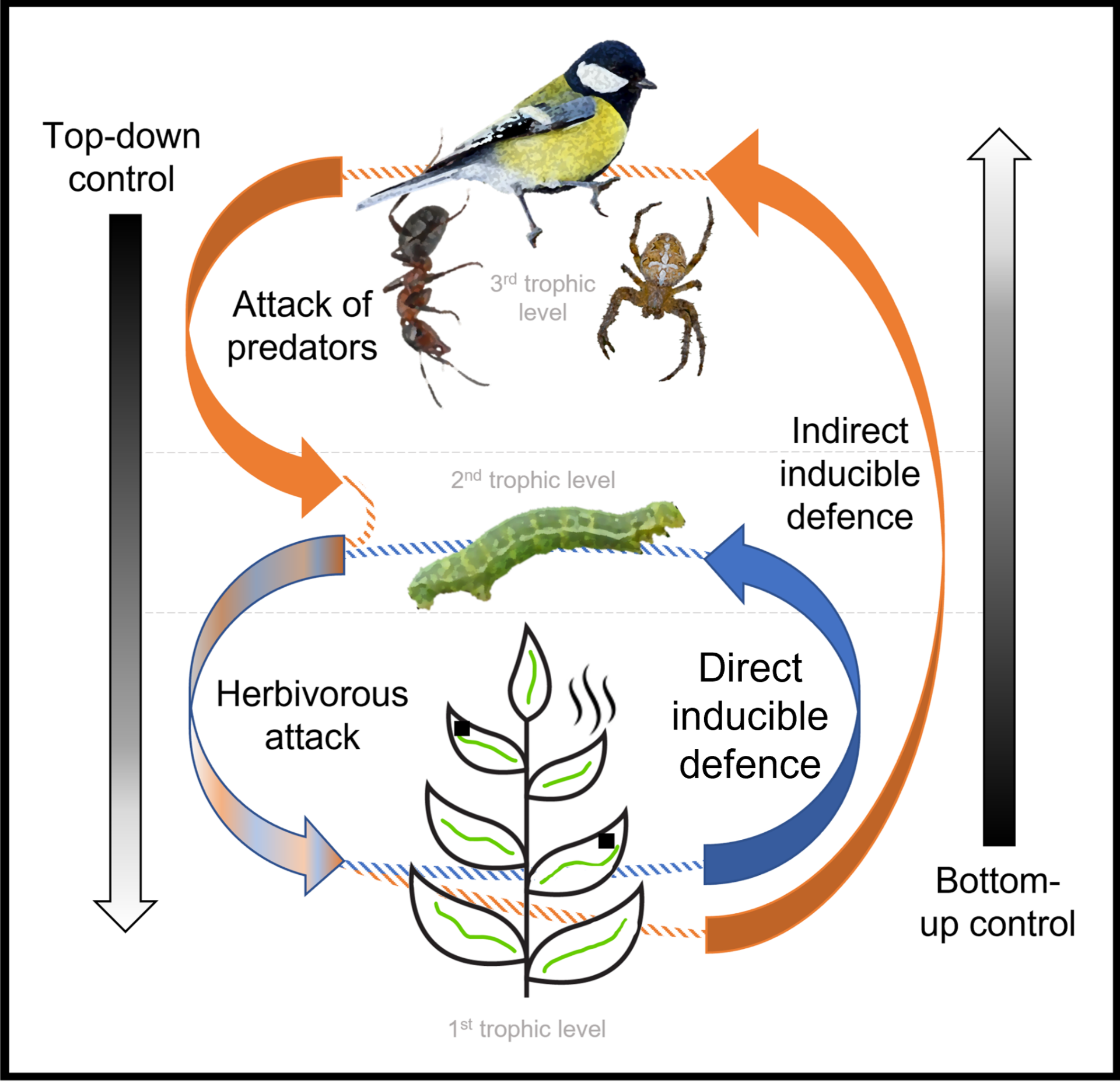
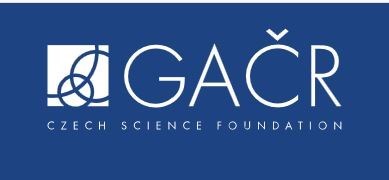
Manipulative Experiments: Trees, Arthropods, Predators
The aim of this project is to understand all components of the plant defences contributing to the bottom-up and top-down mechanisms governing the shape of trophic cascades linked to the English oak across a latitudinal gradient. To achieve this, I proposed an innovative interdisciplinary approach using experimental manipulation of English oak defensive mechanisms and an extensive survey of the majority of trophic interactions affected by them. This pan-European manipulative experiment will test variability in chemical plant defences across a latitudinal gradient under natural conditions and the response of predators and herbivores to them.
If you find the METAP Project meaningful and important (as we do!), fill in a simple form at the bottom of the page and register as a collaborator. Or send us an email to metap.eu@gmail.com.
For now, you can think about it while reading the background and the objective of the project.
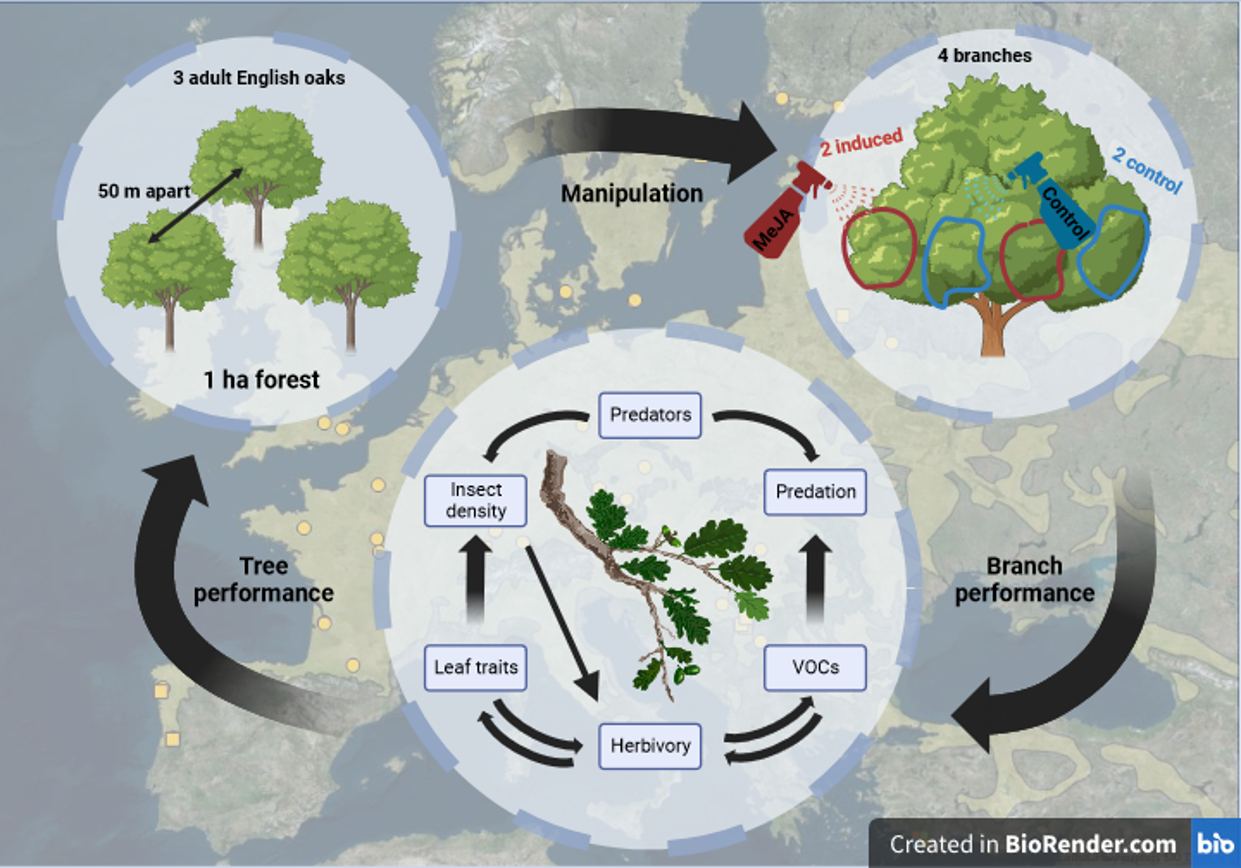
Background
While the nutrients contained in leaves and plant defences determine the abundance of herbivorous insects feeding on plants from the bottom, predators and parasitoids, in turn, affect herbivorous insects negatively from the top [1]. Bottom-up and top-down forces interact passively and actively. Actively, plants exert indirect control upon arthropods by releasing chemical defence compounds which attract predators [1]. Thus, trophic cascades are controlled by plants through communication across trophic levels and plants act as mediators of both, bottom-up and top-down forces acting upon herbivore insects, and other arthropods. It is assumed, that the interplay between top-down and bottom-up interactions varies depending on the ecological context (temperature, humidity, soil characteristics). In the changing world, context is oscillatory, leading to potential disruptions of the mechanisms that plants use to defend themselves, which remain broadly unstudied. Without an understanding of how relatively important are the bottom-up and top-down forces and what is the role of individual types of plant defences them under specific environmental conditions, we cannot predict how the trophic interactions will look in a changing world.
Plants interact with other levels of the trophic cascade through constitutively expressed mechanisms [2] which can be mechanical or chemical or induced upon attack. Chemical inducible plant defences act directly by the synthesis of secondary metabolites making the leaf tissue unpalatable or poisonous [e.g., 2, 3, 4] or indirectly by emitting volatile organic compounds trackable by various predatory taxa [e.g., 5 - 9]. Inducible chemical reactions are regulated by an octadecanoid-based signalling pathway involving the wound hormone, jasmonic acid. The application of jasmonates (jasmonic acid or its volatile derivative methyl jasmonate) in low concentrations to plant leaves has been shown to induce defensive responses [10].
Latitudinal gradients are often used as imperfect proxies of environmental gradients, such as temperature and precipitation. Yet, the climate-latitude relationship can be obscured by geographical constraints and several authors reported that climate predicts the strength of biotic interactions better than latitude per se [11, 12, 13]. Biotic and abiotic factors have been shown to influence plant primary and secondary metabolite profiles along geographic gradients [14]. Constitutive defences trade-off with induced defences and these defensive strategies are expected to relate to growth rate [15]. Slow-growing species invest more in constitutive defence as their biomass is costly and replaceable with difficulties, while fast-growing species invest more in inducible defence [16].
Simultaneous consideration of bottom-up and top-down forces could allow a complex understanding of the patterns of tri-trophic interactions varying along large-scale gradients, which might contribute to the understanding of the behaviour of the cascades under different climatic conditions.
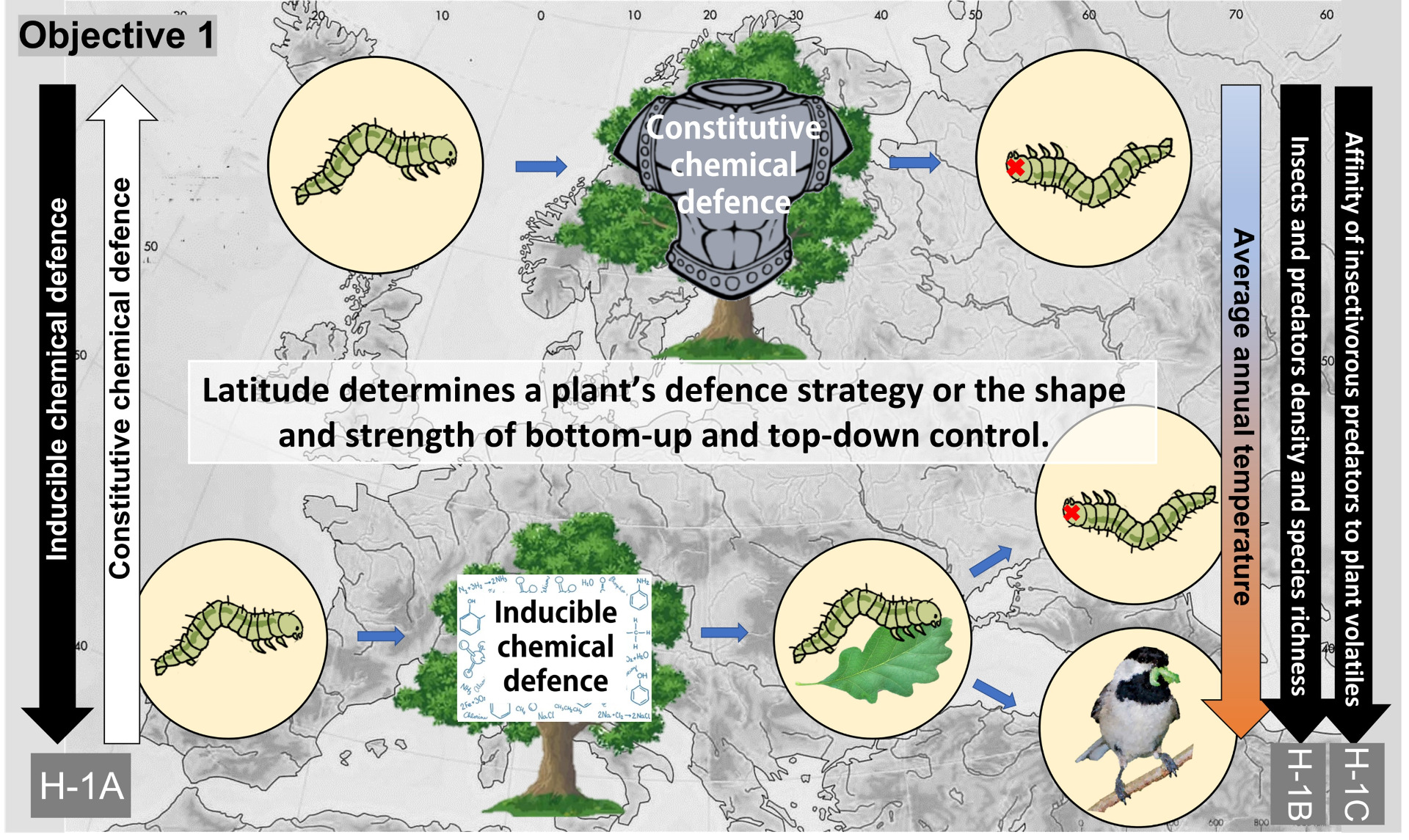
Research objective
Characterise latitudinal clines in plant defence induction and indirect defences.
Is latitude an all-encompassing factor determining plant defence strategy?
If so, the trade-off between chemical constitutive and chemical inducible defence may be detectable along the gradient. According to our preliminary results, tannins (part of the chemical constitutive defence) are more concentrated in English oak in the North [12]. In collaboration with scientific partners from more than 50 sites in Europe, I will conduct a simply designed field manipulative experiment with artificial induction of defence response in trees to test whether (1A) the importance of inducible plant chemical defences is negatively correlated with latitude and thus with the constitutive defences. Inter alia, this hypothesis is also supported by the fact that plant biomass in the north is less regenerative [17], and grows slowly [e.g., 18, 19]; the absolute abundance and diversity of insect predators are higher in the south [11], as is herbivore pressure [20]. Further, the effectiveness and functioning of indirectly induced chemical defences are entirely dependent on predator communities. In case of the absence of predators, there would be no one to respond to the induced compounds. Thus, I predict that (1B) density and species richness of both insect and predator communities negatively correlates with latitude. The inducibility of trees is closely related to the 'Cry for help' hypothesis [21, 22]. Predators of herbivorous insects use volatile organic compounds by the induced plant to locate foraging herbivorous insects. The reaction of birds and other insectivorous predators to herbivore-induced defences. I hypothesise that (1C) the affinity of insectivorous predators to plant volatiles released by the induced plant is negatively correlated with latitude.
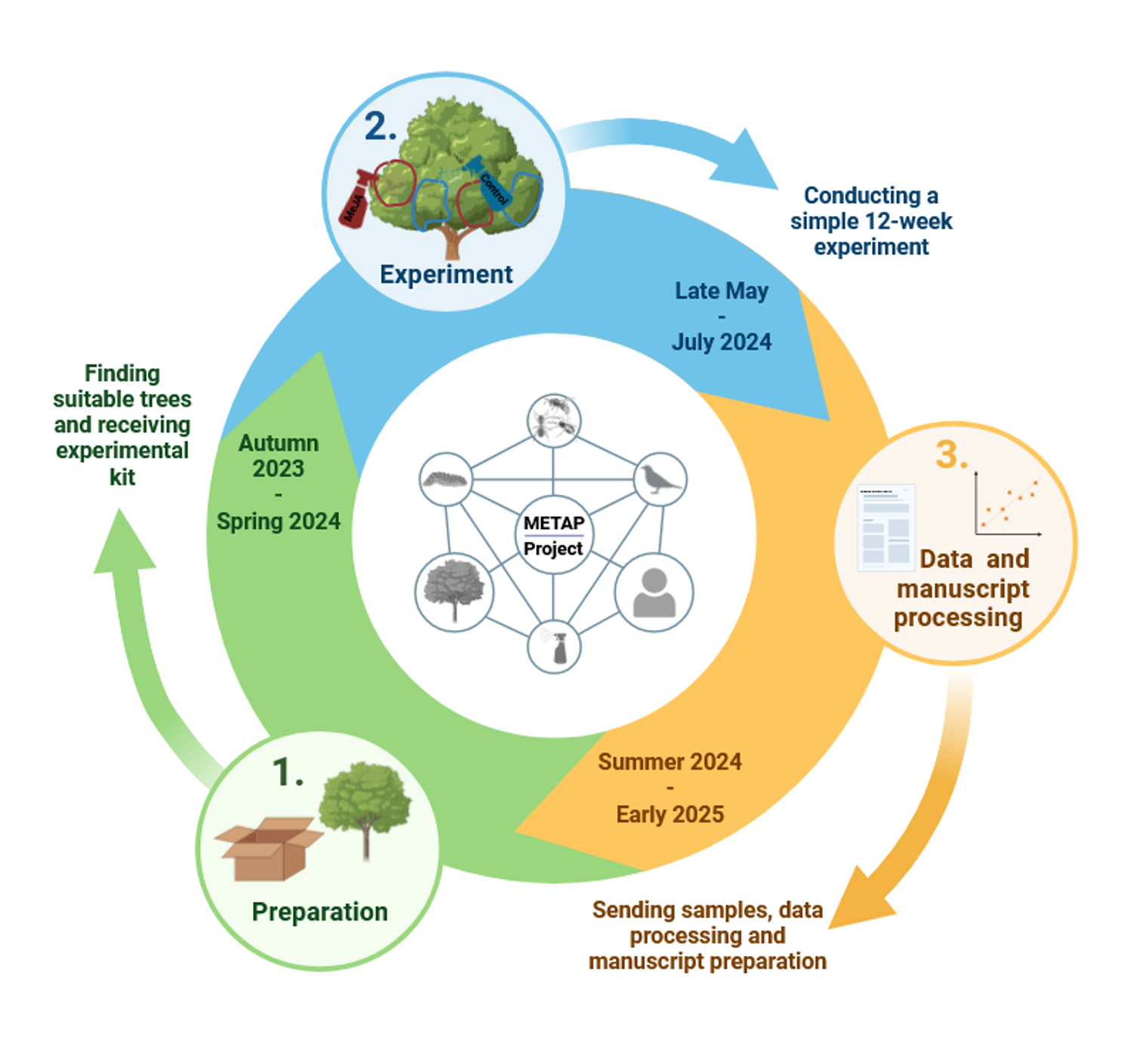
References
1. K. A. Mooney et al., Proc. Natl. Acad. Sci. U. S. A., 107 (16), 7335-7340 (2010).
2. M. S. Chen, Insect Sci., 15(2), 101-114 (2008).
3. A. Mithöfer, W. Boland, Annu. Rev. Plant Biol., 63, 431-450 (2012).
4. M. Dicke, J. J. A. val Loon, Nature Chem. Biol., 5, 317-324 (2009).
5. M. Dicke, R. M. P. van Poecke, J. G. de Boer, Basic Appl. Ecol., 4 (1), 27-42 (2003).
6. M. Heil, New. Phytol., 204 (2), 297-306 (2014).
7. A. Mrazova, K. Sam, Arthropod Plant Interact., 12 (1), 1-8 (2018).
8. A. Mrazova, K. Sam, J. Trop. Ecol., 35 (4), 157-164 (2019).
9. A. Mrazova, K. Sam, L. Amo, Curr. Opin. Insect. Sci., 32, 131-136 (2019).
10. J. S. Thaler, et al., J. Chem. Ecol., 22 (10), 1767-1781 (1996).
11. T. Rostlin, et al., Science, 356 (6339), 742-744 (2017).
12. E. Valdés-Correcher, et al., Glob. Ecol. Biogeogr., 30 (3), 651-665 (2021).
13. M. Mullin, et al., J. Chem. Ecol., 47 (3), 280-293 (2021).
14. A. Kempel, et al., Proc. Natl. Acad. Sci. U. S. A., 108 (14), 5685-5689 (2011).,
15. X. Moreira, et al., Ecol. Lett., 17 (5), 537-546 (2014).
16. L. Chen, et al., Proc. Natl. Acad. Sci. U. S. A., 110, E1963-E1971 (2013).
17. P. De Frenne, et al., Ann. Bot., 109 (5), 1037-1046 (2012).
18. D. B. Botkin, J. F. Janak, J. R. Wallis, J. Ecol, 60, 849-872 (1972).
19. D. L. Urban, M. E. Harmon, C. B. Halpern, Clim. Change, 23, 247-266 (1993).
20. D. Salazar, R. J. Marquis, Proc. Natl. Acad. Sci. U. S. A., 109 (31), 12616-12620 (2012).
21. I. T. Baldwin, J. C. Schultz, Science, 221, 277-279 (1983).
22. D. F. Rhoades, Am. Chem. Soc. Symp., 208, 55-68 (1983).